A collection of interesting kids story books, download and read free children's story books pdf. Choose different the free children's story books online and read wide ranges of books from graphic picture books to short story books as well. All the latest reviews, trailers, release dates, news, screenshots, walkthroughs, and more for Slide Stories: Bonds & Memories.
Our editors will review what you've submitted and determine whether to revise the article.
Join Britannica's Publishing Partner Program and our community of experts to gain a global audience for your work!Slide Stories: Bonds & Memories Cracker Barrel
Thomas O. MasonCeramic composition and properties, atomic and molecular nature of ceramic materials and their resulting characteristics and performance in industrial applications.
Industrial ceramics are commonly understood to be all industrially used materials that are inorganic, nonmetallic solids. Usually they are metal oxides (that is, compounds of metallic elements and oxygen), but many ceramics (especially advanced ceramics) are compounds of metallic elements and carbon, nitrogen, or sulfur. In atomic structure they are most often crystalline, although they also may contain a combination of glassy and crystalline phases. These structures and chemical ingredients, though various, result in universally recognized ceramic-like properties of enduring utility, including the following: mechanical strength in spite of brittleness; chemical durability against the deteriorating effects of oxygen, water, acids, bases, salts, and organic solvents; hardness, contributing to resistance against wear; thermal and electrical conductivity considerably lower than that of metals; and an ability to take a decorative finish.
In this article the relation between the properties of ceramics and their chemical and structural nature is described. Before such a description is attempted, though, it must be pointed out that there are exceptions to several of the defining characteristics outlined above. In chemical composition, for instance, diamond and graphite, which are two different forms of carbon, are considered to be ceramics even though they are not composed of inorganic compounds. There also are exceptions to the stereotypical properties ascribed to ceramics. To return to the example of diamond, this material, though considered to be a ceramic, has a thermal conductivity higher than that of copper—a property the jeweler uses to differentiate between true diamond and simulants such as cubic zirconia (a single-crystal form of zirconium dioxide). Indeed, many ceramics are quite conductive electrically. For instance, a polycrystalline (many-grained) version of zirconia is used as an oxygen sensor in automobile engines owing to its ionic conductivity. Also, copper oxide-based ceramics have been shown to have superconducting properties. Even the well-known brittleness of ceramics has its exceptions. For example, certain composite ceramics that contain whiskers, fibres, or particulates that interfere with crack propagation display flaw tolerance and toughness rivaling that of metals.
Slide Stories: Bonds & Memories Cracking
Nevertheless, despite such exceptions, ceramics generally display the properties of hardness, refractoriness (high melting point), low conductivity, and brittleness. These properties are intimately related to certain types of chemical bonding and crystal structures found in the material. Chemical bonding and crystal structure are addressed in turn below.
Chemical bonds
Underlying many of the properties found in ceramics are the strong primary bonds that hold the atoms together and form the ceramic material. These chemical bonds are of two types: they are either ionic in character, involving a transfer of bonding electrons from electropositive atoms (cations) to electronegative atoms (anions), or they are covalent in character, involving orbital sharing of electrons between the constituent atoms or ions. Covalent bonds are highly directional in nature, often dictating the types of crystal structure possible. Ionic bonds, on the other hand, are entirely nondirectional. This nondirectional nature allows for hard-sphere packing arrangements of the ions into a variety of crystal structures, with two limitations. The first limitation involves the relative size of the anions and the cations. Anions are usually larger and close-packed, as in the face-centred cubic (fcc) or hexagonal close-packed (hcp) crystal structures found in metals. (These metallic crystal structures are illustrated in Figure 1.) Cations, on the other hand, are usually smaller, occupying interstices, or spaces, in the crystal lattice between the anions.
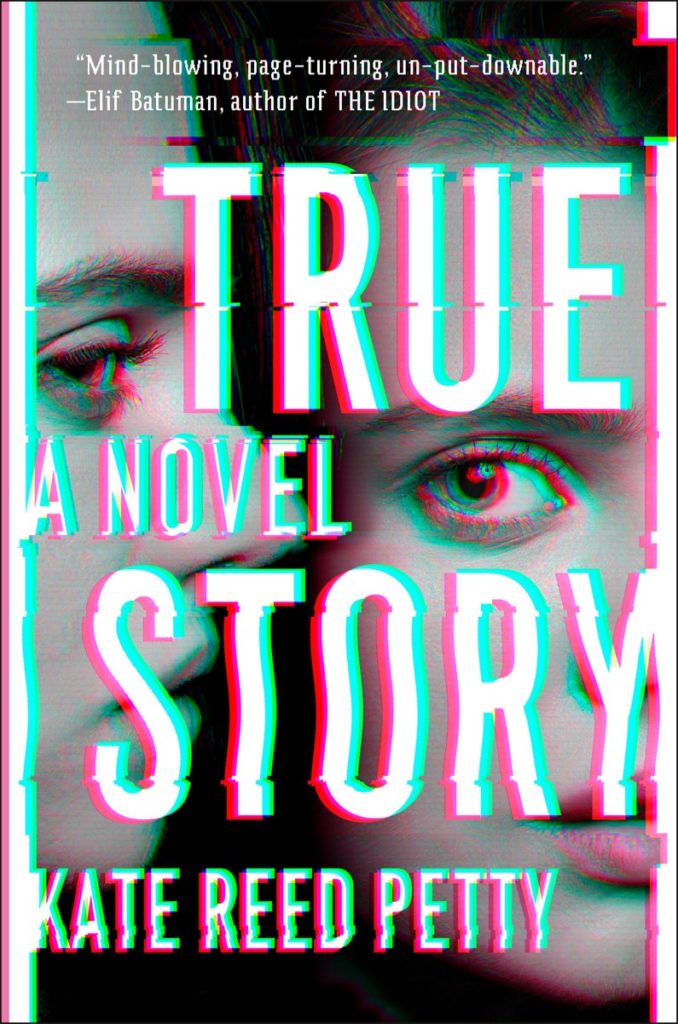

The second limitation on the types of crystal structure that can be adopted by ionically bonded atoms is based on a law of physics—that the crystal must remain electrically neutral. This law of electroneutrality results in the formation of very specific stoichiometries—that is, specific ratios of cations to anions that maintain a net balance between positive and negative charge. In fact, anions are known to pack around cations, and cations around anions, in order to eliminate local charge imbalance. This phenomenon is referred to as coordination.
Most of the primary chemical bonds found in ceramic materials are actually a mixture of ionic and covalent types. The larger the electronegativity difference between anion and cation (that is, the greater the difference in potential to accept or donate electrons), the more nearly ionic is the bonding (that is, the more likely are electrons to be transferred, forming positively charged cations and negatively charged anions). Conversely, small differences in electronegativity lead to a sharing of electrons, as found in covalent bonds.
Secondary bonds also are important in certain ceramics. For example, in diamond, a single-crystal form of carbon, all bonds are primary, but in graphite, a polycrystalline form of carbon, there are primary bonds within sheets of crystal grains and secondary bonds between the sheets. The relatively weak secondary bonds allow the sheets to slide past one another, giving graphite the lubricity for which it is well known. It is the primary bonds in ceramics that make them among the strongest, hardest, and most refractory materials known.
Crystal structure
Crystal structure is also responsible for many of the properties of ceramics. In Figures 2A through 2D representative crystal structures are shown that illustrate many of the unique features of ceramic materials. Each collection of ions is shown in an overall box that describes the unit cell of that structure. By repeatedly translating the unit cell one box in any direction and by repeatedly depositing the pattern of ions within that cell at each new position, any size crystal can be built up. In the first structure (Figure 2A) the material shown is magnesia (MgO), though the structure itself is referred to as rock salt because common table salt (sodium chloride, NaCl) has the same structure. In the rock salt structure each ion is surrounded by six immediate neighbours of the opposite charge (e.g., the central Mg2+ cation, which is surrounded by O2− anions). This extremely efficient packing allows for local neutralization of charge and makes for stable bonding. Oxides that crystallize in this structure tend to have relatively high melting points. (Magnesia, for example, is a common constituent in refractory ceramics.)
The second structure (Figure 2B) is called fluorite, after the mineral calcium fluoride (CaF2), which possesses this structure—though the material shown is urania (uranium dioxide, UO2). In this structure the oxygen anions are bonded to only four cations. Oxides with this structure are well known for the ease with which oxygen vacancies can be formed. In zirconia (zirconium dioxide, ZrO2), which also possesses this structure, a great number of vacancies can be formed by doping, or carefully inserting ions of a different element into the composition. These vacancies become mobile at high temperatures, imparting oxygen-ion conductivity to the material and making it useful in certain electrical applications. The fluorite structure also exhibits considerable open space, especially at the centre of the unit cell. In urania, which is used as a fuel element in nuclear reactors, this openness is believed to help accommodate fission products and reduce unwanted swelling.
The third structure (Figure 2C) is called perovskite. In most cases the perovskite structure is cubic—that is, all sides of the unit cell are the same. However, in barium titanate (BaTiO3), shown in the figure, the central Ti4+ cation can be induced to move off-centre, leading to a noncubic symmetry and to an electrostatic dipole, or alignment of positive and negative charges toward opposite ends of the structure. This dipole is responsible for the ferroelectric properties of barium titanate, in which domains of neighbouring dipoles line up in the same direction. The enormous dielectric constants achievable with perovskite materials are the basis of many ceramic capacitor devices.
The noncubic variations found in perovskite ceramics introduce the concept of anisotropy—i.e., an ionic arrangement that is not identical in all directions. In severely anisotropic materials there can be great variation of properties. These cases are illustrated by yttrium barium copper oxide (YBCO; chemical formula YBa2Cu3O7), shown in Figure 2D. YBCO is a superconducting ceramic; that is, it loses all resistance to electric current at extremely low temperatures. Its structure consists of three cubes, with yttrium or barium at the centre, copper at the corners, and oxygen at the middle of each edge—with the exception of the middle cube, which has oxygen vacancies at the outer edges. The critical feature in this structure is the presence of two sheets of copper-oxygen ions, located above and below the oxygen vacancies, along which superconduction takes place. The transport of electrons perpendicular to these sheets is not favoured, making the YBCO structure severely anisotropic. (One of the challenges in fabricating crystalline YBCO ceramics capable of passing large currents is to align all the grains in such a manner that their copper-oxygen sheets line up.)